Electrode Materials for Better EV Battery Performance
Battery electrode materials face significant challenges in maintaining structural integrity and performance over thousands of cycles. Current lithium-ion electrodes experience volume changes exceeding 300% during cycling, leading to mechanical degradation and capacity loss. Surface-level decomposition and internal structural changes occur at operating voltages above 4.3V, particularly affecting nickel-rich cathode materials.
The fundamental challenge lies in balancing high energy density with long-term structural stability while maintaining economically viable manufacturing processes.
This page brings together solutions from recent research—including surface modification techniques for improved stability, three-dimensional electrode architectures, composite materials with engineered porosity, and novel synthesis methods for manganese-based oxides. These and other approaches focus on practical implementations that can scale to mass production while meeting increasingly demanding performance requirements.
1. Method for Synthesizing Silicon Monoxide Composite with In-Situ Grown Carbon Nanotubes
Hong Zhao, 2024
A method for preparing a silicon monoxide composite material with improved electrical conductivity and initial coulombic efficiency compared to pure silicon monoxide for use in lithium-ion batteries. The method involves growing carbon nanotubes directly on the surface of the silicon monoxide using an in-situ catalyst. This provides a uniform carbon coating with nanotubes and a small amount of amorphous carbon that improves electrical conductivity and reduces volume variations during charging/discharging compared to adding carbon externally.
2. Three-Dimensional Lithium Anode with Vertical Columnar Structure and Conformal Capping Layer on Copper Substrate
Oerlikon Surface Solutions AG, Pfäffikon, 2024
Three-dimensional lithium anode for high-capacity lithium-ion batteries that addresses the limitations of graphite anodes. The anode has a vertical structure with columnar or grid-shaped lithium deposited on a copper substrate. A conformal capping layer is deposited over the lithium to protect it and prevent dendrite growth. The vertical structure allows higher lithium loading density compared to flat graphite anodes. The capping layer prevents volume expansion and ensures stable cycling. The 3D lithium anode has higher capacity, lower weight, and better cycling compared to graphite anodes.
3. Layered Positive Electrode Active Material with Surface-Enriched Additive Element X for Structural Reinforcement
SEMICONDUCTOR ENERGY LABORATORY CO., LTD., 2024
A positive electrode active material for lithium-ion batteries that retains its structure and capacity after repeated charge/discharge cycles. The material has a surface region with higher concentration of an additive element X compared to the interior. This reinforces the outer surface and prevents breakage of the layered structure as lithium is extracted during charging. The higher X content surface helps the material maintain its structure and capacity over cycles compared to a homogeneous composition.
4. Anode Structure with Fluoropolymer Coating Layer for Inhibiting Binder Decomposition in Lithium-Ion Batteries
Hyundai Motor Company, Kia Corporation, SEOUL NATIONAL UNIVERSITY R&DB FOUNDATION, 2024
Anode design for lithium-ion batteries with reduced binder decomposition during cycling. The anode has a coating layer between the active material core and the binder. The coating layer is made of a fluoropolymer like PVDF, PTrFE, PCFE, or PCTFE. This coating blocks electron transport from the core to the binder, preventing decomposition of the binder during cycling.
5. Composite Oxide with Tetragonal Structure for Lithium-Ion Battery Anodes
KABUSHIKI KAISHA TOSHIBA, 2024
Active material for high capacity lithium-ion batteries with improved initial charge-discharge efficiency and cycling performance. The active material is a composite oxide with a tetragonal crystal structure represented by the general formula LiaTibNb2-2dMc+2dO2b+5+3c, where M is W or Mo and the subscripts satisfy certain constraints. This composite oxide provides high charge/discharge capacity per volume compared to traditional carbon anodes. It shows favorable initial efficiency and cycling stability in lithium-ion batteries.
6. Porous Battery Active Material via Li Extraction from Li22Si5 Crystal Phase
TOYOTA JIDOSHA KABUSHIKI KAISHA, 2024
Reducing volume change of battery active materials during charging/discharging by creating a porous structure inside the material particles. The method involves preparing a LiSi precursor with a specific crystal phase, then extracting Li using a solvent to form voids in the precursor. This step creates a porous active material with reduced volume change compared to non-porous materials. The specific crystal phase is Li22Si5.
7. Electrode Assembly with Low Thermal Conductivity Barriers for Enhanced Thermal Runaway Resistance
CONTEMPORARY AMPEREX TECHNOLOGY CO., LIMITED, 2024
Electrode assembly for secondary batteries with improved thermal runaway resistance to prevent battery failures in abuse situations. The electrode assembly has barriers made of current collectors with low thermal conductivity in at least one electrode plate. The barrier thickness and thermal conductivity satisfy a condition of lambda over delta less than 3x107 W/(K*m2) to provide effective thermal resistance. This impedes heat spreading if a local failure occurs, reducing risk of chain reactions and thermal runaway.
8. Manganese Oxide Compositions with Lithium and Sodium for Battery Electrodes
Honda Motor Co., Ltd., 2024
Manganese oxides for lithium-ion and sodium-ion batteries with high capacity, long cycle life, and low cost. The manganese oxides have compositions containing lithium and/or sodium, like Li1.5Mn0.5O2 or Na1.5Mn0.5O2. They can be synthesized by introducing manganese, sodium, and metal precursors under specific conditions. The metal can be any element except manganese or sodium. The resulting oxides have improved performance compared to conventional manganese oxides used in batteries.
9. Carbon-Based Materials with High Surface Area and Variable Pore Size via Modified and Non-Hummers' Methods
The Regents of the University of California, 2024
Carbon-based materials, fabrication methods, and energy storage devices with improved performance. The carbon materials have high surface area, pore size, and oxygen content. They can be made using a modified Hummers' method or a non-Hummers' method. The modified Hummers' method involves cooling the graphite and sulfuric acid mixture before oxidation. The non-Hummers' method involves adding potassium permanganate, agitating, cooling, and hydrogen peroxide. These materials can be used as active materials in lithium-ion battery electrodes, coating on lithium metal negative electrodes, or asymmetric supercapacitor electrodes.
10. Lithium Iron Phosphate Cathode with Vanadium and Optional Cobalt Co-Doping for Enhanced Power Density and Moisture Resistance
A123 Systems LLC, 2024
Lithium-ion battery cathode material with improved power density and reduced moisture uptake for low-voltage applications like electric vehicles. The cathode is lithium iron phosphate (LFP) with specific dopant compositions and synthesis methods to avoid NH3 emissions and achieve target properties like high capacity, rate capability, and low temperature performance. The LFP formulation has a molar ratio of phosphate to iron around 1.00-1.05, vanadium dopant partially replacing Fe, and total non-lithium metal to phosphate ratio around 1.00-1.04. The vanadium dopant is VPO4, which has similar anions to FePO4, for higher efficiency. Optionally, cobalt co-doping is used. The synthesis involves mixing the dopants, iron phosphate, l
11. Layered Crystal Structure Cathode Material with Surface-Enriched Transition Metals for Lithium-Ion Batteries
KABUSHIKI KAISHA TOSHIBA, 2024
An active material for lithium-ion battery cathodes that improves cycle life and capacity retention compared to traditional cathode materials. The active material has a layered crystal structure with a composition of Li(Ni, Co, Mn, Mg, Al, K, Na, Ca, Si, Ti, V)O2 where some of the transition metal elements (Nb, Ta) are disproportionately enriched at the surface. This suppresses side reactions and oxygen loss during cycling, reducing capacity fade. The active material also has a specific surface area of 5-50 m2/g. The enriched surface transition metals prevent surface layer formation and improve cycle life compared to traditional cathode materials.
12. Negative Electrode with Micron-Scale Particle Protective Layer for Lithium Metal Batteries
SAMSUNG ELECTRONICS CO., LTD, 2024
Negative electrode for lithium metal batteries with improved cycle life and reduced volumetric change during charging. The negative electrode has a protective layer on the lithium metal surface with particles sizes between 1-100 microns. The protective layer has a Young's modulus of 106 Pa or greater. This provides mechanical strength to prevent dendrite growth and volumetric expansion during charging. The protective layer also improves lithium deposition density compared to bare lithium metal electrodes.
13. All Solid State Battery with Selective Anode Coating for Controlled Resistance Distribution
TOYOTA JIDOSHA KABUSHIKI KAISHA, 2024
An all solid state battery design to prevent short circuits in the anode during charging by controlling the resistance distribution. The battery has a coating layer with lithium titanate on the anode current collector. The coating exists in the region where the anode and cathode are opposing but is omitted in the region where they are not opposed. This helps balance charge reaction progression in both regions. In the opposed region, the coating provides a conductive path to lower anode potential. In the non-opposed region, the coating omission reduces resistance compared to the coated region. This prevents uneven charge reaction progression and minimizes short circuits in the anode.
14. Composite Anode Material with Dendritic and Non-Porous Group 4A Nanoparticles Forming Porous Agglomerates
GEORGIA TECH RESEARCH CORPORATION, 2024
Anode material for lithium-ion batteries that addresses the limitations of pure silicon anodes in improving battery performance. The anode is a composite made of agglomerated nanocomposites where each nanocomposite has a dendritic nanoparticle of silicon or other Group 4A element surrounded by discrete non-porous nanoparticles of the same element. The dendritic nanoparticles interconnect in the agglomerated nanocomposites to form a porous structure. This allows volume expansion of the silicon during charging without separating from the current collector. The composite anode also has improved electrical conductivity compared to pure silicon.
15. Electrode Assembly with Circumferential and Radial Slits for Enhanced Electrolyte Distribution and Contact Area
LG ENERGY SOLUTION, LTD., 2024
Electrode assembly design for batteries to improve electrolyte impregnation and reduce internal resistance. The electrode has slits in the uncoated portions along the circumference and radial direction. These slits allow electrolyte to pass through and uniformly fill the electrode stack. The slits also provide wider contact areas when bent to secure the electrode tabs. This reduces internal resistance and improves coupling strength compared to unslotted electrodes.
16. Lithium Electrode with Dual-Layer Composite Protective Coating for Dendrite Inhibition
LG ENERGY SOLUTION, LTD., 2024
Lithium electrode for batteries with a protective layer to prevent dendrite growth in lithium metal anodes. The protective layer is a composite of two layers: a first layer close to the lithium metal with high ion conductivity, and a second layer further from the lithium metal with high electrical conductivity and mechanical strength. The first layer allows lithium ions to pass and prevents lithium depletion. The second layer transfers electrons to the lithium surface and prevents localized current density. The composite layer structure inhibits dendrite growth and improves battery performance compared to single-layer coatings.
17. Composite Active Material with Nb2TiO7 and Nb-rich Phases for Lithium-ion Battery Negative Electrodes
KABUSHIKI KAISHA TOSHIBA, 2024
Active material for high-performance lithium-ion battery negative electrodes that balances capacity, cycle life, and energy density. The active material contains both Nb2TiO7 and Nb-rich phases like Nb10Ti2O29, Nb14TiO37, and Nb24TiO64. It also has optimized particle size distribution and contains potassium and phosphorus. The Nb-rich phases improve overcharge resistance and cycle life. The potassium and phosphorus help suppress particle growth during synthesis. The particle size distribution is fine enough for good rate performance but not excessively small to prevent cracking during cycling.
18. Lithium-Ion Battery Cathode Additives Comprising Mn+1AXn Compounds for Manganese Dissolution Mitigation
Rivian IP Holdings, LLC, 2024
Additive materials for lithium-ion batteries that prevent or reduce manganese dissolution during charging/discharging cycles. The additive materials are compounds with the general formula Mn+1AXn, where M is an early transition metal, n is 1-3, A is a group 13/14 element, and X is C or N. These MAX compounds have improved thermodynamic stability compared to manganese and can be added to lithium-ion battery cathodes to decrease manganese dissolution in the electrolyte. This improves battery performance by preventing capacity loss and structural changes caused by manganese leaching.
19. Cylindrical Secondary Battery with Specific Electrode Uncoated Shape and Segmented Bent Structure for Current Path Optimization
LG ENERGY SOLUTION, LTD., 2024
Cylindrical secondary battery with reduced internal resistance and improved energy density compared to conventional tab-less batteries. The battery has an electrode assembly where the uncoated portions of the positive and negative electrodes have a specific shape and current path ratio. The uncoated portions have sections that are not used as tabs. The maximum current path ratio through these sections is limited to 11 or less. This prevents excessive resistance buildup when welding the current collectors. The limited ratio allows minimizing resistance while still having large capacity. The battery also has a segmented bent structure for the uncoated portions. This allows overlapping and stacking the segments to provide a wider area for welding the collectors.
20. Lithium Battery with Lithium-Silicon Composite Electrode and Protective Layer
BYD COMPANY LIMITED, 2024
Lithium battery with high energy density and improved cycle life by using a lithium-silicon composite negative electrode. The battery has a lithium-silicon composite negative electrode active material with elemental lithium and a lithium-silicon alloy. The battery also has a protective layer on the negative electrode to suppress side reactions and lithium plating. During charging, the battery is stopped at a lower cutoff voltage where no lithium is deposited on the negative electrode. This prevents dendrite formation and improves cycle life.
Get Full Report
Access our comprehensive collection of 997 documents related to this technology
Identify Key Areas of Innovation in 2025
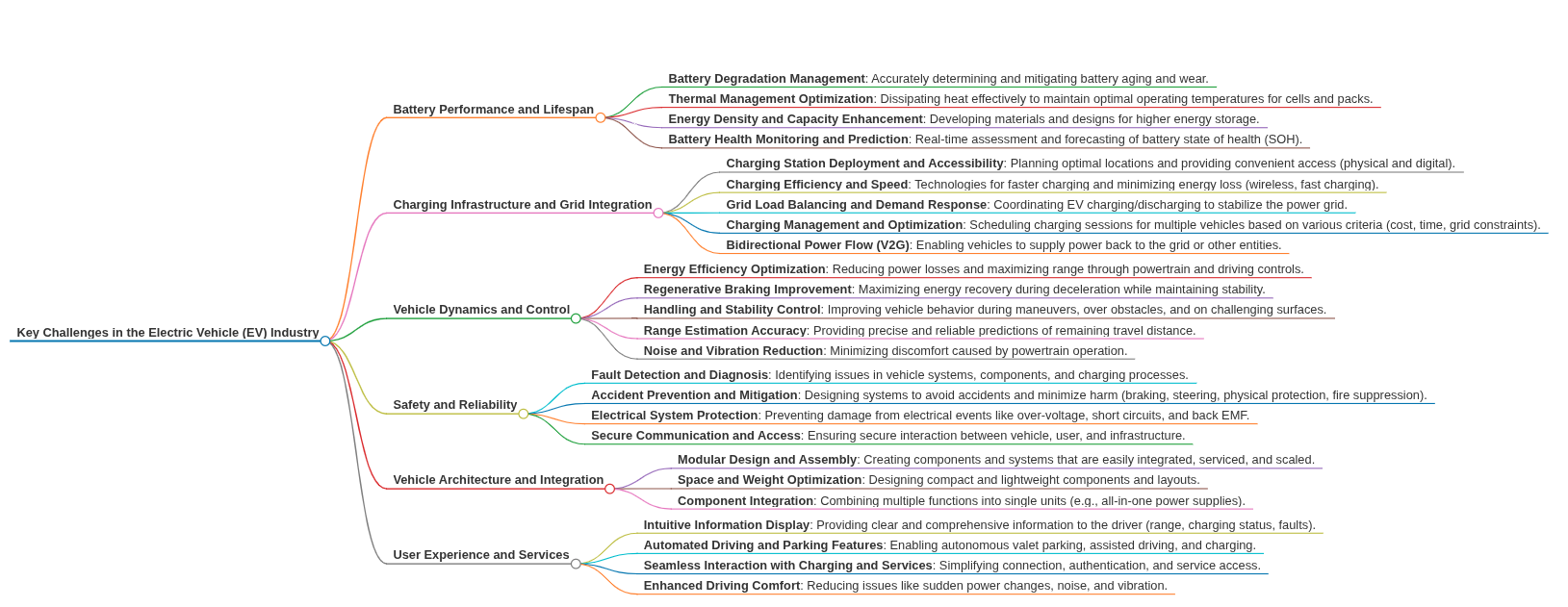